Introduction.
In the previous post, I covered some issues in defining addiction and also presented current thinking about the role of the brain’s reward circuitry in addiction development. While the reward circuitry, prefrontal cortex, and amygdala are clearly involved , I suggest here that isn’t the whole story. In this post, I present the hypothesis that the unreasoning and lasting need of an addict may be strongly influenced by neural circuitry located in the more primitive parts of our brain.
Wanting vs Liking a Drug.
Intuitively, you might think that wanting a drug and liking a drug are flip sides of the same coin. However, their paradoxical dissociation in drug addicts might provide a clue as to where the critical addiction circuitry is located. For example, compulsively wanting a drug is lowest when you first use the drug and increases as addiction sets in. When wanting a drug reaches some intensity, we would say the person is addicted. At the same time, drug addicts will tell you that a drug is most pleasurable in the early days of use, while pleasure tends to dull with continued use. This inverse relationship (very roughly schematized below) suggests that the cause of wanting a drug is not the same as that of liking a drug.

We know a great deal about the neuroanatomy of “liking.” Liking is related to activation of the reward circuitry which allows the amygdala to assign hedonic value to stimuli and behaviors. This information can then can be made available to the the prefrontal cortex for use in conscious thought processes and behavior.
Addiction as maladaptive learning?
However, to understand the neuroanatomy of addiction, the neural circuitry underlying “wanting” is most critical. Wanting should be guided by brain areas that encode “reward expectancy”. According to this explanation, you should want to encounter stimuli or perform behaviors associated with an expectation of reward and avoid those associated with an expectation of adverse outcomes. Reward expectancy is normally acquired by associative learning through repeated experiences with a particular situation. For example, learning to want a stimulus is typically acquired through Classical (or Pavlonian) Conditioning while learning a desired appetitive behavior (that provides access to a reward) is learned through Operant (or Skinnerian) Conditioning.
Addiction is a relatively permanent change in behavior brought about by experience (which also happens to be the classical definition of learning). In fact, some experts now think that addiction is a form of maladaptive learning in parts of the brain that encode “reward expectancy.” However, the learning is so powerful that once acquired, it overrides all other expectancies. The addict can’t seem to help herself even though she often knows better.
So, what areas of the brain encode reward expectancy? There is some evidence that the cingulate gyrus (a part of the cerebral cortex) in its interactions with the prefrontal cortex plays such a role in humans. By occurring in a part of the brain that can be consciously accessed, such expectancies provide us with a conscious knowledge of what we like and dislike. However there is a problem for conscious reward expectancies being solely responsible for encoding the compulsive desires of addicts. As the drug becomes less rewarding and the increase in adverse consequences enter the addict’s consciousness, drug use should begin to extinguish. (This doesn’t mean that the original expectancy is being forgotten, but rather that new competing knowledge should keep the original expectation from being acted upon). In contrast, despite liking the drug less and suffering increasingly negative consequences, many addicts intensify their drug use.
This outcome suggests that the circuitry underlying reward expectancies may not be entirely in conscious areas of the brain. I don’t consider myself a Freudian, but I do think Freud got at least one thing right: there are a lot of important things that occur below the level of conscious awareness over which we humans have relatively little control.
Evolution of the unconscious and conscious parts of our brains.
In what follows I give you my simplified “big picture” of human brain evolution to provide some background as to where the “addiction circuitry” might be found.
The brain began as an enlargement of the upper part of the spinal cord to provide executive control over the rest of the nervous system and ultimately the body. Natural selection favored this change because it led to better adapted and more reproductively fit organisms. The primitive brain not only detected sensory inputs and directed motor outputs, it also optimized homeostatic processes critical to life such as breathing, heart rate, swallowing, blood pressure etc. And since associative learning is a fundamental property of ALL nervous tissue, the primitive brain was capable of using simple stimulus/response learning to connect sensory inputs to motor outputs. Consciousness had not yet evolved, so all these processes were below the level of conscious awareness. Thus, the capabilities of the primitive vertebrate brain (as seen in a fish) would, for the most part, appear innate, reflexive, and hard-wired.
A general evolutionary principle is that any genotype or trait that makes a big contribution to adaptation and reproductive success tends to be conserved (i.e. doesn’t change much) over evolutionary time. While the human brain has diverged significantly from that of fish, the organization of their brainstems (hindbrain, midbrain, and posterior part of the forebrain) has remained remarkably similar. All the same areas are represented, and for the most part, are doing the same sorts of things. If you understand the anatomy of the fish brainstem, you also know a great deal about the anatomy of the human brainstem. Clearly mother nature did an excellent job in designing this structure and has not made dramatic changes from fish to human. However, the anterior part of the mammalian forebrain was selected by natural selection to go in a new and very different direction. This part of the brain not only became much larger, more complicated, and added new functionality, it also provided the basis for the evolution of mammalian consciousness.
Mammalian consciousness reaches its zenith in the human cerebral cortex due, in some unknown way, to its large size, greatly increased storage capacity, highly interconnected circuitry, and much more complicated executive functioning by the prefrontal cortex. The prefrontal cortex is the part of the brain that you “think” with. It makes conscious decisions about what you should be doing and when you should be doing it. The prefrontal cortex uses sensory input as well as information that it has stored elsewhere in the cortex to aid in making decisions. At the same time, for the prefrontal cortex to be consciously aware of something, that something must be represented by neural activity within the cortex itself. While the cortex may be vaguely aware of the brain’s unconscious activities, it doesn’t know details. For example, while your cortex knows you can ride a bike, it doesn’t know which muscles need to be contracted or relaxed, and in what sequence. Those “motor melodies” are encoded in an unconscious part of the brain (the cerebellum).
At the same time, new cortical tissue was added over time in what appears to be a modular fashion. The new conscious processing did not replace the older unconscious processing, it was in addition to the older processing. As a result, there is some redundancy in the conscious processing of the cortex and unconscious processing of the lower brain. However, neural connections between them normally allows for adaptive coordination.
While the highly modified mammalian forebrain (i.e. cortex, limbic system, basal ganglia, and the fiber tracts that interconnect them) expanded behavioral flexibility and promoted broader adaptation, the more primitive brainstem remained tightly linked to unconscious homeostatic processes such as breathing, blood flow, body temperature regulation, etc. In the unusual situation where higher and lower brain areas would be in conflict regarding these critical processes, the lower brain areas should normally win out. For example, it’s virtually impossible to commit suicide by consciously holding your breath. No matter how hard you try, the unconscious breathing centers in the medulla (lowest part of the brain) will make you start breathing again. (Hopefully nobody reading this proves me wrong!) It is also instructive to look at the effects of brain damage. Because the medulla is in charge of homeostatic processes critical to life, damage to the primitive medulla is much more likely to be lethal than damage to the much more complicated cortex.
Conflicts arising from independent processing in different parts of the brain.
While independent processing in different parts of the brain usually works together, it is possible to have conflicts. Some of the best examples are found in split-brain preparations where the corpus callosum (the fiber tract that connects the two cortical hemispheres) has been cut (typically to treat epilepsy), causing the 2 hemispheres to be disconnected in their functioning. When this happens, the two hemispheres are less able to coordinate their processing and occasionally bizarre outcomes can occur where one hemisphere will try to produce one set of motor responses while the other hemisphere will try to produce another. More important to the addiction argument would be conflicts between higher and lower brain areas. While higher and lower brain areas also usually work together, conflicting processing should be possible here as well.
A good example of independent processing by higher and lower brain areas is the brain’s processing of visual information. As seen in the figure below, our conscious visual experience is begun by processing occurring in the primary visual cortex of the occipital lobe. However, a lower part of the human brain (the superior colliculus in the midbrain) is also independently processing visual input, but below the level of conscious awareness. In fact, for vertebrates that lack a neocortex (such as reptiles and birds), the optic tectum (equivalent to the human superior colliculus) is the primary visual processing center.

Visual processing by the superior colliculus is thought by some to to explain another bizarre phenomenon in humans known as “blindsight”. Blindsight occurs following brain damage to the primary visual cortex in the occipital lobe of the cortex (necessary for conscious visual processing). Such a person would be diagnosed as blind by an ophthalmologist. However, if the eyes, the optic nerve, and visual pathways into the lower parts of the brain including the superior colliculus are undamaged, such a “blind” person can still behaviorally respond to certain types of visual input. However, when asked what caused the response and why they responded, the person cannot give a good explanation. A commonly accepted explanation is that the visual input is being processed by the superior colliculus operating below the level of conscious awareness.
While both the human visual cortex and superior colliculus normally work together, their independent visual processing is thought to serve different purposes. While the much more sophisticated cortical processing influences conscious thought and behavior, the simpler superior colliculus processing may be important for quick reflexive responses to visual input. We do know that the human superior colliculus does process visual input to influence several different reflexive eye and head movements known to facilitate vision. However, whether the superior colliculus underlies blindsight is based more upon indirect evidence. Like the primary visual cortex, a retinotopic map exists in the superior colliculus where specific locations in the superior colliculus correspond to particular locations in the retina. The relationship suggests that the human superior colliculus might have the neural machinery to reconstruct some representation of what the person is seeing. The superior colliculus also is connected to the spinal cord by a fiber tract (the tectospinal pathway) that could mediate quick output to human body muscles as it does in birds and reptiles. This role also makes anatomical sense because the superior colliculus both receives visual information sooner and, can reflect it back out to muscles faster than the cortex, allowing for quick (but unconscious) visual reflexes.
The point of this digression is that while different parts of the brain normally work together, independent processing of similar information does occur at different brain levels. And should conflicts arise concerning issues important to survival and reproductive fitness, the lower brain areas should sometimes have priority. And few things are more important to survival than quickly identifying and responding to rewarding (and aversive) stimuli.
So where is the addiction circuitry?
I offer the hypothesis (not original with me) that addiction may be influenced by memory circuitry somewhere in the unconscious parts of the brain relatively immune to the influence of conscious learning. Exactly where is a question I won’t try to answer. However, I would suggest that the lower this circuitry is in the brain, the more resistant it would be. I will now weasel out of the situation by hoping that future research will be able to address this very important question. I will also leave it to persons smarter than me to figure out what, if any, implications this could have for addiction treatment.
As I pointed out in the first post on addiction, the prevailing school of thought is that addiction can be accounted for by dysfunctions in the reward circuitry as well as in the prefrontal cortex and amygdala. I am not discounting the important role these forebrain structures play, particularly in encoding the initial conscious reward expectancies. However, I am suggesting as addiction proceeds, the forebrain dysfunctions (particularly in the prefrontal cortex) may contribute even more by impairing the organized conscious thought processes necessary to override powerful primeval reward expectancies unconsciously encoded the brainstem.
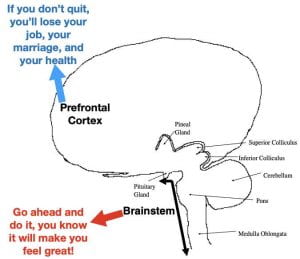
The reality is that we don’t really completely understand what encodes and maintains the need to engage in this highly compulsive, long-term, out-of-control behavior and all explanations (including the one presented here) should be taken with a grain of salt.
Sorry to take so long to reach such an unsatisfying conclusion. 🤷♂️But…….that’s the way science sometimes goes! Still a lot to learn!
To learn more.
Advocat, Comaty & Julien (2019). Chapter 4: Epidemiology and neurobiology of addiction. In Julien’s Primer of Drug Action. Thirteenth Edition. Worth Publishers. 267-295. (A good textbook that I last used in my teaching. many references to primary literature at the end of this chapter)
Charles P. O’Brian. Drug use disorders and addiction. Chapter 24 in Goodman and Gilman’s The pharmacological basis of therapeutics. McGraw-Hill Publishers. (written for medical professionals so fairly technical)
I also refer you to Wikipedia for information on addiction, split-brain preparations, blindsight, and consciousness. The Wikipedia entries also provide citations to the primary literature.
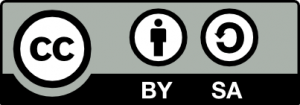